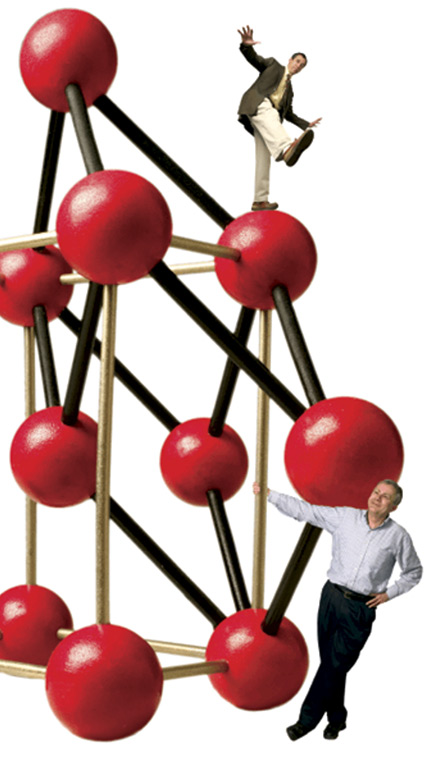
With the help of an $11.4 million grant from the National Science Foundation, Penn’s new Nano/Bio Interface Center is asking the “fundamental questions” about nanotechnology.
By Samuel K. Moore | Photography by Bill Cramer
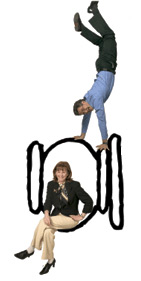
Here’s a design task for the next decade: Start with a truck. Now, take away the driver and the fuel tank; then make it small enough to fit inside the slender branches of a human brain cell, smart enough to pick up the right sack of mood-regulating chemicals, and powerful enough to haul its cargo from one side of the brain to the other. Oh yes, and it has to assemble itself and operate perfectly in the equivalent of hurricane-force winds, too.
Machines like these, which would have to be comprised of one or just a few individual molecules, have been the decades-long dream of scientists and engineers working in the field of nanotechnology, and making them will push engineering to its limits.
Up until very recently even the most advanced microchips followed physical rules that remained largely true regardless of how big or small the device was. But in the realm of nanotechnology, where parts have dimensions measured in the millionths of a millimeter, everything is different. The color of a speck of gold is not gold but red or green depending on the speck’s exact size and shape; vibrations act like particles; motion is not smooth but full of seemingly random jiggles. In short, the rules change, and we don’t know most of them.
The good news is that life figured these rules out a long time ago. The proof that nature solved the aforementioned miniature truck problem, for example, is the billions of molecular motors crawling around in every cell of your body right now. The hope is that if we can learn the rules by which natural electrical, optical, and mechanical molecular devices work, we will not only get a better grasp of physiology but one day design our own molecular devices.
At Penn, that effort was given a major boost last fall when the University was named one of the six new Nanoscience and Engineering Centers (NSECs) to be funded by the National Science Foundation. Penn received a five-year grant of $11.4 million, renewable to $23 million, to study the interface of nanotechnology and biology at the molecular level. In November, the Nano/Bio Interface Center (NBIC) was set up to provide a home for a diverse group of Penn scientists and engineers working on various aspects of this effort. With other existing grants, about $30 million in funding will support Penn’s nanotechnology research efforts.
The six NSECs funded in this round of grants join eight others established since 2001. Each center has a different mission that complements its home institution’s strengths. Penn’s research on the interface of nanotechnology and biology at the molecular level could lead to advancements in nanoscale device manufacturing, drug delivery, and integrated chemical sensors, as well as an increased understanding of basic complex biological and physiological processes.
“Penn has tremendous strengths in biomedical research and also the physical sciences,” says NBIC Director Dawn Bonnell, a professor of materials science in the School of Engineering and Applied Science. “In fact, of all the NSECs funded so far, none of them are able to bridge this gap between the physical sciences and biology. So [NSF is] very excited about this one in particular.” Scientists and engineers from 10 departments across the University, including arts and sciences, the medical school, and five of the six engineering disciplines, will take part.
While some other NSECs are geared toward commercially oriented science in particular fields such as silicon microchips or magnetic storage media, Penn’s mission is both more broad and more purely scientific. “We’re asking the fundamental questions,” says Bonnell. “We see ourselves, in a very broad sense, as enabling nanotechnology.”
An important part of enabling nanotechnology will be helping society determine rules governing its use. Penn’s cadre of noted bioethicists, including Dr. Arthur Caplan, the Hart Professor of Bioethics and chair of medical ethics and director of the Center for Bioethics, and Dr. Paul Root Wolpe C’78, professor of psychiatry and senior faculty associate at the Center for Bioethics, will help shape the discussion of the ethical impact of nanotechnology.
The key to most of the research in the NBIC is the ability to observe and manipulate single molecules. “It’s only been fairly recently that we’ve been able to look at single molecules,” Bonnell explains. In fact, some say nanotechnology was born with this ability. That came with the invention of the scanning tunneling microscope (STM). This tool, invented in the 1980s at IBM’s Zurich laboratory, earned its creators the Nobel Prize in physics. By scanning a tiny, sharp-tipped probe just above a conductive surface and measuring the weak current that resulted, the STM allowed scientists for the first time to look at and even push around individual atoms. The original model was followed by a variant called the atomic force microscope, and now there exists a bestiary of derivations able to resolve a host of physical properties and manipulate molecules on a surface. Bonnell’s laboratory is responsible for one such device, the scanning impedance microscope, which can actually show nanometer-scale defects in a material where electrons can get stuck.
Biophysics, too, has recently developed tools to study single molecules, and NBIC collaborator Dr. Yale Goldman, professor of physiology and director of the Pennsylvania Muscle Institute, has been using them to uncover fundamental rules about how natural molecular motors work. There are three main types of molecular motors in human cells, and they are responsible for a wide variety of tasks. What they have in common is the ability to translate chemical energy into mechanical forces.
Goldman’s most lauded recent success centers around a class of molecular motors called myosins. Most myosins can be found in muscle inching along a partner molecule called actin. Here myosin forms a thick filament with many protruding arms. When a muscle contracts, the myosin grabs hold of the actin and pulls.
But there are some 20 other kinds of myosin and these do all manner of molecular chores. In immune cells called leukocytes they help the cell to change shape, making it seem to crawl after invading microbes. In brain cells they carry microscopic sacks filled with chemical transmitters such as serotonin from the cell’s body out along its trunk-like axon where it connects to other brain cells.
Goldman’s lab has put particular emphasis on myosin V, a version of the motor that looks a bit like the bottom half of a stick figure. The molecule has two long leg-like chains that come complete with “feet” that interact with long filaments of actin, which act as molecular highways within a cell. Whatever molecular cargo the cell needs moved around attaches to the top of this myosin, and once it finds an actin filament it will move long distances without leaving the road. Defects in myosin V are implicated in a very rare but potentially fatal childhood neurological, immune, and pigment disorder called Griscelli syndrome.
Scientists were puzzled for a long time as to how this motor actually walks on its actin trail. They knew it would have to keep at least one foot attached to the actin at all times or else it would just float away. But does it move by shuffling along, with one foot stepping forward and the other one coming up to meet it? Does it pivot like a cartographer’s compass? Does it walk in a one-foot-in-front-of-the-other manner? Through some clever molecular biology and the ability to look at one molecule at a time, Goldman helped prove that myosin V does walk one-foot-in-front-of-the-other, or turning the analogy on its head, hand-over-hand. “It’s like a child on monkey bars,” he says. His lab helped solve the problem by watching a single myosin molecule that had been slightly chemically modified by adding a molecule called a fluorophore to one of its legs. When hit by a certain color of light fluorophores glow. Watching the way the fluorophore moved indicated the hand-over-hand motion.
Just adding a fluorophore by itself is not enough to let you see one molecule’s motion, though—particularly if you are looking for motion that’s on the order of only a few dozen nanometers at a time. The glow from a single molecule is swamped by the background light of dust and other contaminants. So Goldman’s group turned to one of the relatively new technologies that have let scientists begin to probe the goings-on of individual molecules. They managed to cut the background glow down by about 1,000-fold using what’s called total internal reflectance (TIRF) microscopy. In TIRF you shine a laser at a very shallow angle on a sample of molecules on a glass slide. The laser light reflects within the glass, leaving only a small amount just at the surface, called effervescence, to make fluorophores there glow.
“When we study individual molecules we are able to get the kind of information that we were never able to find with ensemble experiments,” says Goldman.
Experiments on large batches of molecular motors could show that myosin V moved along actin without falling off, but not much else. But beyond proving how myosin V walks, single-molecule techniques showed that the molecule was subject to what Goldman calls “a seemingly chaotic and violently harsh environment.” Water molecules are knocking the molecular motor around millions of times each second, but far from keeping the motor from doing its job, it appears that the tiny machine is designed for such abuse.
Goldman has an animation of what myosin V actually looks like during a typical step. While about two thirds of the machine’s step is purposefully powered by its chemical motor, the last third is the result of random motions driven by collisions with water. The stick figure almost looks drunk as it tries to complete a step by placing its foot on the actin. Understanding how a natural molecular machine not only survives but thrives in all that random motion is crucial to the future of nanotechnology. Technologists have long envisioned making machines out of single molecules that can do coordinated work. Nanotechnology visionary K. Eric Drexler popularized the idea in the late 1980s, thinking that an army of such molecular machines could in theory make any other machine using just atoms as raw materials. “This is the real environment that Drexler’s little machines would have to operate in, and it’s not intuitive,” says Goldman. “But we can understand it by studying these biological machines.
“We’re using nanotechnology to learn about biophysical processes that happen in your body—and perhaps we’ll use those to engineer devices—but we’re asking the fundamental questions,” he says. “When the community in general gets to the point where they are going to start making devices out of molecular motors, if these fundamental questions haven’t been answered, they’ll hit a wall.”
While Goldman is writing the rules of mechanics of individual biomolecules, another NBIC team co-led by Dr. Alan T. “Charlie” Johnson, associate professor of physics and astronomy, and Dr. Haim Bau, professor of mechanical engineering and applied mechanics, is figuring out the laws that govern their electrical and optical properties. “We hope to figure out what the rules are and use those rules to make more complicated systems,” says Johnson.
Specifically, they are trying to understand the properties of artificial proteins. Proteins are chain-like molecules that fold into particular shapes to act as enzymes, structural components, hormones, and much of everything else in living things. In order to be able to do all those things, they have the capacity to be fantastically complex. And it is this complexity that attracted Johnson to study proteins. In fact, he wanted to get started studying the electro-optical properties of proteins when he first arrived at Penn in 1994. But the theoretical understanding and physical tools needed had not yet been developed. And another molecule came calling: the carbon nanotube. These hollow tubes of crystalline carbon measure just over a nanometer in diameter but can be several thousand times that size in length. They have become the darling of the nanotechnology world because of their unheard-of mechanical and electronic properties: they are stronger than steel but lighter than aluminum, they can act as either conductive wires or semiconductors like those that make up computer chips, they can transport current or heat along their length with little resistance, they can even be made to emit light.
As fascinating a material as carbon nanotubes may be, they are quite simple when compared to proteins, says Johnson. And the NBIC researchers are able to take advantage of their understanding of nanotubes. “We’re building on our knowledge of electronics at the molecular scale, except we want to work with some molecules that are more complicated than carbon nanotubes,” says Johnson. “It’s an idea whose time has come from a physics standpoint.”
The proteins Johnson is working with are chains consisting of any of 20 biochemicals called amino acids. The sequence in which the amino acids occur determines what shape the chain folds into, and that shape determines many of its properties. In particular it determines what other chemicals, called cofactors, can be attached to it and where they fit. An example of a protein and its cofactors is hemoglobin, where the protein carries the cofactor heme, which does the work of carrying oxygen in blood. The sequence of amino acids that make up proteins in the NBIC experiments do not correspond to anything found in nature. Instead they are designed to hold cofactors—not all of them are found in nature, either—in particular spots and at particular orientations in the protein. While the proteins are important too, the cofactors are the heart of the molecule’s electro-optical properties.
Johnson’s optoelectronics experiments are a perfect example of interdisciplinary science. Biophysics Professor William DeGrado designs the proteins based in part on computational theory by Associate Professor of Chemistry Jeffery G. Saven. The cofactors are designed by Chemistry Professor Michael J. Therein. Using his own techniques and some tools developed in Dawn Bonell’s lab, Johnson puts the molecule on a chip between two electrodes that are separated by just a few nanometers and measures how current flows through it both in the presence and absence of light.
Being able to measure a single molecule at a time lets scientists observe the effects of subtle things in the molecule’s environment. You can see, for instance, the effect of the kind of metal the electrodes are made of, the influence of a nearby molecule, or the importance of the material on which the protein is lying.
Bonnell points out, too, that unless you look at the individual molecule, you can’t resolve the problems posed by the environment or discover some unexpected but useful physics that can be exploited. A circuit being influenced by something in its environment in a predictable way is the very definition of a sensor, and Johnson thinks sensor design is one discipline that could benefit from the work his group at NBIC is doing.
Whatever physical rules NBIC researchers come up with, whether and how they are used will be up to the rules set forth in public policy. Since there are precious few nanotechnology-based products on the market or even in late-stage development, it might seem that having an ethical discussion about them is premature. But Arthur Caplan can already identify several emerging ethical issues, and there are likely to be more. The best way to identify them and perhaps shape their outcome, he says, is to work closely with the people who are pushing the technology forward, such as NBIC researchers. “This is one of the first times we’ve got a handshake going on between bioethics and engineering,” Caplan says, adding that now is the time to get these issues squared away—before nanotechnology presents any real-life ethical dilemmas. “The best bioethics is prophylactic bioethics,” he says. He and his colleagues have already come up with several categories of ethical issues that the use of nanotechnology faces.
The first is dealing with the fear that some form of nanotechnology will somehow escape the bounds of the environment it was designed for and cause harm. In its most imaginative form, this is the scenario described in Michael Crichton’s recent science-fiction novel, Prey, in which a “grey goo” of self-replicating, nanometer-scale robots breed with abandon and turn on their creators. But Caplan and others do not see this as a serious threat. Even the coiner of the “grey goo” term, K. Eric Drexler, dismisses the danger. “Runaway replication would only be the product of a deliberate and difficult engineering process, not an accident,” he wrote in the August 2004 issue of the journal Nanotechnology. The popular identification of nanotechnology with grey goo is hurting public perception of the field and distracting attention from more serious and realistic safety concerns, Drexler added.
Nanotechnology does not have to crawl off and commit cold-blooded murder to be a danger. Although research on health effects of nanoscale materials is just getting started, some of the most promising nanoscale materials have been found to be harmful in certain situations. Two reports in 2004 stirred concern. One showed that high concentrations of carbon nanotubes can fatally stop up the lungs of rats. The other found that fullerenes, spherical carbon cages just a few nanometers across, tend to accumulate in fish brains. Nanotechnology industrialists, particularly those involved in manufacturing nanoscale materials, are concerned that more such studies could cause a public backlash. “It’s a bit like the worries about agricultural biotechnology,” notes Caplan. “Nanotech watched what happened to agricultural biotech and almost had a heart attack.”
Another ethical issue the NBIC is tracking is the question of whether the use of nanotechnology in our bodies will make us somehow less human, more like a cyborg. Already, people are volunteering to have rice-grain sized radio-frequency ID tags injected beneath their skin to serve as identification for calling up medical records or entering secure areas. Nanotechnology could make such devices much smaller and more intelligent. And what issues would arise about the expectation of privacy if such an unobtrusive device could be used to keep tabs on people without their knowledge?
There are also ethical issues associated with intellectual property rights. Caplan is concerned as to whether the public interest is best served by issuing patents describing broad science or technology or by issuing only narrow ones that leave the broad concepts in the public domain or even in government hands. At this early stage in the development of nanotechnology some very fundamental patents have already been assigned, though their impact is unclear. For instance, computing and software giant, IBM Corp., owns the U.S. composition of matter patent on the type of carbon nanotube most likely to make it into practical applications in electronics and materials.
Right now, NBIC research is done in the home labs of its investigators, but building a home for the center is a top priority for both the School of Engineering and Applied Science and the University. For SEAS it will be the last and most expensive piece in an infrastructure trifecta that has so far seen the completion of a new information technology building, Levine Hall, and the continuing construction of a biotechnology building, Skirkanich Hall. “We knew nanotech would be the toughest one to get done,” says SEAS Dean Eduardo Glandt GCh’75 Gr’77.
“For IT you need desks and computers, for biotech you need wet labs, but for nanotech you need clean rooms,” he says. Found in microchip factories, the environment in a clean room is so tightly controlled that in the most advanced type only one particle of dust will be found for every cubic foot of air. On top of clean rooms, portions of the laboratory must be isolated from the imperceptible microscopic vibrations caused by the world outside.
It’s a demanding set of criteria. Bonnell points out that the particles in smoke are about 10,000 times bigger than some of the molecules NBIC scientists work with, and that the vibrations in her office in the Laboratory for Research on the Structure of Matter building are just as comparatively enormous. “You don’t always have to have such control [for nanotechnology experiments] but your building has to accommodate it.”
So critical has nanotechnology become that she estimates 35-40 percent of research projects in applied physics and engineering requires the conditions the NBIC will provide. “[Nanotechnology] is not a flash-in-the-pan topic. It really represents the fundamental underpinning of a wide range of academic disciplines,” she says. And if the center produces the research she expects, Penn science and engineering will be the fundamental underpinnings of the nanotechnology era.
Samuel K. Moore is an associate editor with IEEE Spectrum Magazine and writes frequently on nanotechnology issues.