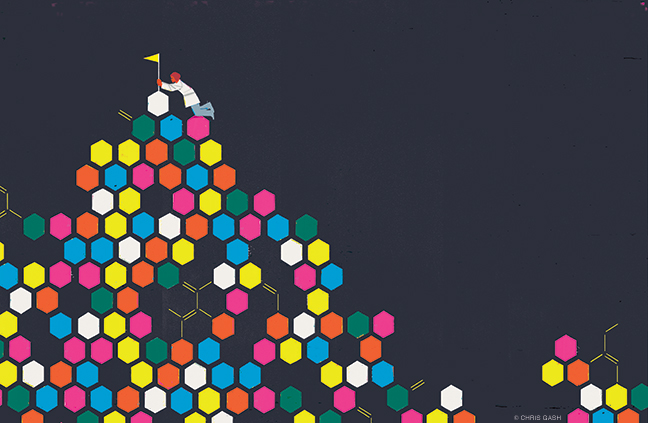
From matchbook-sized models of living human organs to the surprising alternative-energy implications of symbiotic giant clams, the work of three new faculty members represents the changing face of bioscience at Penn.
BY TREY POPP
Illustration by Chris Gash
Photography by Candace diCarlo
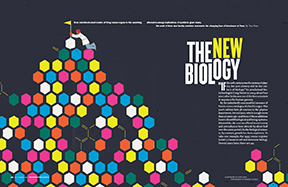
“If the 20th century was the century of physics, the 21st century will be the century of biology.” So proclaimed biotechnologist Craig Venter in 2004, about four years after he became one of the first scientists to sequence the human genome.
By the (admittedly unscientific) measure of Penn’s course catalogue, it’s hard to argue. This year’s edition lists 58 courses in the physics department, for instance, which is eight more than 20 years ago—and three of those additions have to do with biological and living systems. (Meanwhile, the courses offered in astronomy and astrophysics have shrunk by about half over the same period.) In the biological sciences, by contrast, growth has been explosive. To take one example, the 1995 course register listed 17 classes in cell and molecular biology. Twenty years later, there are 49.
The technological advances fueling this increase are apparent in other departments as well. Today, a bioengineering student can take classes like “Nanoscale Systems Biology,” “Principles, Methods, and Applications of Tissue Engineering,” and “Brain-Computer Interfaces.” Good luck finding any of those things in the 1995 catalogue. The intervening decades have witnessed the maturation of completely new subfields, ranging from epigenetics to computational biophysics.
The growing diversity of course offerings and bioscience research at Penn ultimately derives from faculty, which the University has been recruiting aggressively. From Hayden Hall to the Carolyn Lynch Laboratory, there’s a lot going on—far too much for a comprehensive overview. Or even a non-comprehensive one, for that matter. So in this issue we take a look at three fresh faces helping to redefine life sciences at Penn. Their interests vary as much as their departmental affiliations, but biology is a common factor.
All three are assistant professors who have landed on campus within the last four years. Two were hired in connection with the Evolution Cluster initiative. Led by Randall Kamien, the Vicki & William Abrams Professor in the Natural Sciences, this is a joint effort by SAS faculty to create an academic hub for interdisciplinary researchers seeking a “quantitative understanding of evolutionary processes … across the natural sciences, social sciences, humanities, and computational mathematics.” It charges departments to work together to fill vacancies with collaboration-minded candidates “without any predetermination as to the department in which she or he would sit.” Allison Sweeney, profiled below, was a prototype hire. An evolutionary biologist with a specialty in marine biology, she sits in the Department of Physics and Astronomy. Erol Akçay, another Evolution Cluster member, is a theoretical biologist with a more traditional appointment in the Department of Biology.
Dongeun (Dan) Huh hails from the School of Engineering and Applied Science, where he is the Wilf Family Term Assistant Professor of Bioengineering. Like Sweeney, he is interested in biomimicry—but while she focuses on replicating aspects of a symbiotic relationship between algae and giant clams, he is busy creating micro-engineered models of human organs. In other words, the 21st century is well underway in West Philadelphia.
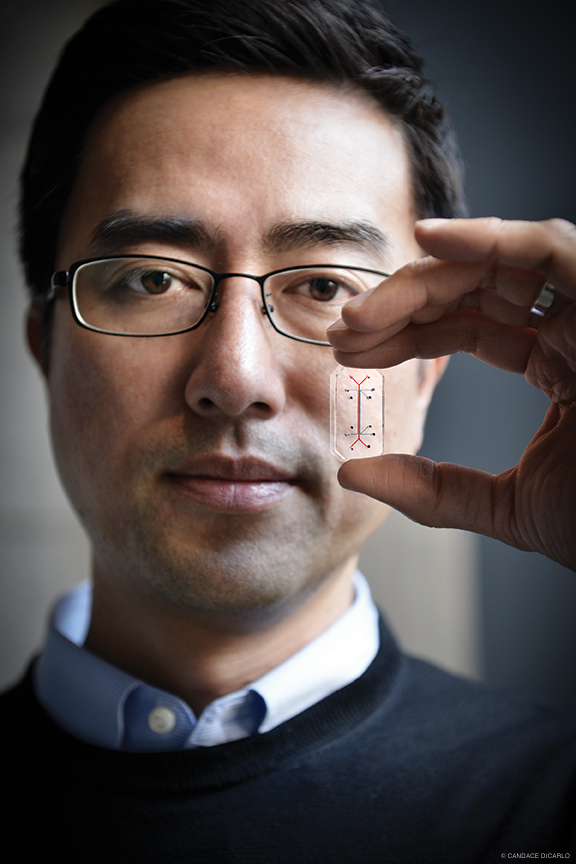
Organs on Chips
Whether you found your calling in a gross anatomy lab or in the science-fiction aisle, you’re liable to feel a little strange when Dan Huh pops open his desk drawer and hands you his lung.
He might tell you what it is at the outset, or he might wait until you’re palming the thing. Either way, it won’t seem right. As small as a matchbox, as smooth as a gummy bear, and as clear as a windowpane except for a simple pair of symmetrically branching red and blue lines, it is the last thing you can imagine coming down with a cough.
But it can. Or at least Huh can give it one—and watch exactly what happens next, in real time. His “lung on a chip” is a living, moving, breathing approximation of a human alveolar air sac.
The average person has about 700 million alveoli; they absorb oxygen and expel carbon dioxide to keep us alive. Huh’s device represents a single sac—or, more accurately, a cross-section of one. It’s made out of the same sort of silicone rubber elastomer used in contact lenses. Those blue and red lines are actually microfluidic channels that run parallel to one another, separated by a very thin, perforated silicone membrane. Huh cultures human lung tissue on one side of the membrane, and flows air over it. On the other side he grows human capillary tissue, and pumps a blood-like liquid under it, as though it were the wall of a blood vessel. A pair of vacuum channels flanks this arrangement; as they expand and contract under pressure, the lung and capillary tissues stretch and contract, mimicking the mechanical motion of breathing.
It’s easy to culture both kinds of cells in a Petri dish. But growing them in this dynamic scaffolding prompts them to behave in a different way—more like how they actually behave in the body.
When exposed to flowing blood-like liquid, the capillary cells elongate and align themselves in the direction of flow, as they do in a human blood vessel. Under air, the lung tissue differentiates into goblet cells, which produce and secrete mucus, and ciliated cells, whose hair-like projections sweep the mucus away along with dust and other unwanted particles. Rooting the lung and capillary tissues in the same perforated silicone membrane—which approximates a web-like structure in the body called the extra-cellular matrix—enables them to work together as they do in a human lung.
Huh, who helped pioneer the device during a fellowship at Harvard’s Wyss Institute for Biologically Inspired Engineering and came to Penn in 2013 from Seoul National University’s College of Medicine, isn’t really interested in breathing. He’s interested in drug discovery and toxicology.
“Pharmaceutical companies are spending more and more money every year to develop new drugs, but the number of new drugs has been declining steadily over the past two decades,” he says.
A 2012 analysis by Forbes concluded that for each new drug the world’s 12 biggest pharmaceutical companies had brought to market over the previous 15 years, between $4 billion and $12 billion had been spent overall on research and development. The main expense is failure: all the money spent producing and evaluating molecules that either fail to work or turn out to be unsafe.
Clinical trials on human subjects are the costliest part of the process, but their high rates of failure—often estimated at around 85 percent—can be traced partly to the limitations of the two primary methods of preclinical testing: in vitro and animal experiments.
“Animal testing that is required for determination of therapeutic efficacy and safety is currently one of the major bottlenecks because it is costly and time-consuming,” Huh has written. “Most worrisome is that traditional animal testing approaches often fail to predict human toxicity.”
To take one example, in 2006 an immunotherapy called TGN1412 that had been found to be safe in rhesus monkeys triggered catastrophic multi-organ failure in human subjects given a dose 500 times smaller than what their primate cousins had tolerated. An academic review of TGN1412’s dangerous failure noted the limitations of animal models and emphasized the need for “in vitro studies on human material as close as possible to the target tissue.”
Organs-on-chips offer an advantage over both classic in vitro methods and animal models: real human tissue, configured to function the way it does in the body.
“We believe that environment is critical,” Huh says. “So as long as we give the cells the right environment, they kind of take care of themselves.” That is, they gain the ability to function in far more complex and lifelike ways than in a Petri dish.
Huh has infected his lung-on-a-chip with fluorescently dyed E. coli bacteria, and watched through a microscope as the lung tissue signaled to the capillary tissue, causing white blood cells to flock to the infection site, squeeze through the interstitial membrane, and engulf the bacteria.
“How our lung fights infection is one of the most complex organ-level physiological responses in the body,” Huh says. “Before this system, people were able to reproduce certain aspects … but showing the entire process, from the bacterial infection all the way to the engulfment, in real time at high resolution like this, [is new].”
He has rigged the device to a cigarette-smoking machine, and shown how it leads to elevated rates of protein-misfolding within cells, and overproduction of proteins that stiffen the extra-cellular matrix in the body, which causes fibrosis. He calls it smoking-lung-on-a-chip, and is interested in finding out whether e-cigarettes have similar effects—an unsettled question with huge public-health implications.
Since coming to Penn, Huh has also developed skin-on-a-chip, placenta-on-a-chip, cervix-on-a-chip, and an eye-on-a-chip—which cries and blinks.
Through academic-pharmaceutical partnerships, as well as a company called Emulate (for which he is a scientific advisor), Huh sees potential for organs-on-chips to help drug companies winnow promising therapeutic compounds.
“They’re desperately looking for next-generation drug-testing platforms,” he says. “They have technologies like genomics technology that are currently allowing them to identify potential drug molecules. And there are so many of them—they are flooded with them. So the challenge is to weed out the drugs that are destined to fail. And that’s where this technology may come in.”
It’s an idea that has spurred interest and research by a number of groups around the country. The last couple of years have brought reports of a variety of microfluidic organs-on-chips, including liver, kidney, heart, and gut. They are feats of design no less than science. In 2015, the lung-on-a-chip Huh helped create was crowned “Design of the Year” by London’s Design Museum. The same year, New York’s Museum of Modern Art acquired a prototype for its permanent collection.
“Right now we’re focused on developing these individual organ models,” Huh says. “But the dream is to link these systems in a physiologically relevant manner, and have them communicate with one another to figure out what’s going on at the whole-body level.”
“A human-body-on-a-chip system would be very exciting.”
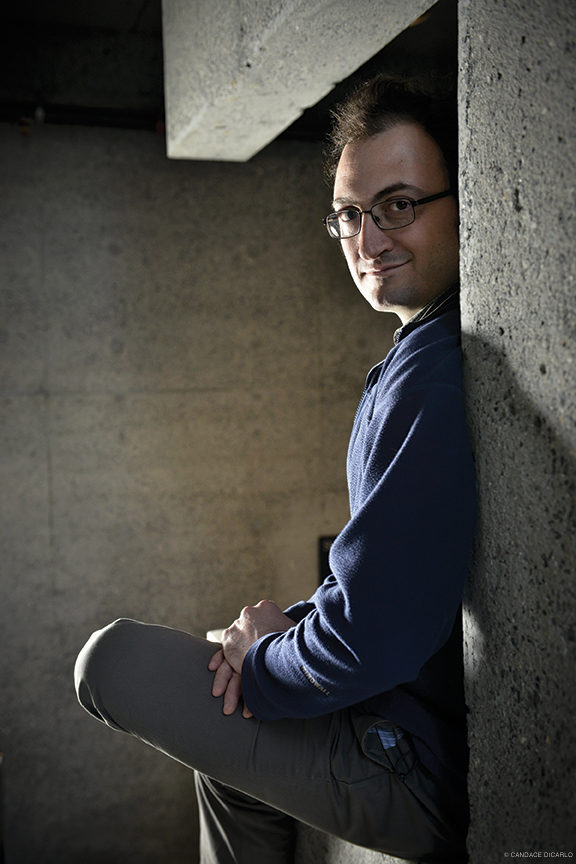
The Biological Game Theorist
Here’s a bit of evolutionary theory that may sound familiar—perhaps so familiar that it seems totally obvious.
Consider the lark bunting, an American sparrow whose males exchange their grey plumage for dramatic black-and-white during the breeding season. Like the vast majority of bird species, lark buntings rear their young by forming socially monogamous male-female pairs. The logistics of the nest illuminate the usefulness of this arrangement. A mother may lay the eggs, but she can’t singlehandedly guard her hatchlings and forage food for them. So having a helpful mate increases the chances for both parties that their progeny will survive. And if the offspring of monogamous couples tend to survive at higher rates, their genetic inheritance will reinforce the species’ tendency toward monogamy.
Yet lark buntings do something else, too: they cheat. That’s a moral term, not a scientific one, of course. But when biologists acquired the tools to conduct avian paternity tests in the 1980s, they found, in one species after another, nests in which females had laid two sorts of eggs: a majority sired by the monogamous social mate, plus a smaller number sired by an absent male.
This wasn’t entirely a shock. In fact, it had been predicted by the reigning intellectual giant of evolutionary biology, Robert Trivers, whose ideas informed some of the the most influential books ever written about the field, including Richard Dawkins’ The Selfish Gene. Trivers explained the phenomenon of “extra-pair paternity” in terms that resonate to this day in popular understandings of evolution. Basically, it goes like this. A mother needs a capable and devoted house husband in order for any of her eggs to survive, but she also has an interest in copulating with the most genetically fit mate possible. And if she can’t land a genetically superior male as a nest mate, she’ll settle for an inferior one, “but then sort of sneak on the side and get the good genes from the outside male,” as assistant professor of biology Erol Akçay puts it.
The idea that sexual selection is at bottom a competitive hunt, in which females vie for the best or most-compatible genes, while males spread their own as widely as their anatomy permits, is powerful. It has driven a great deal of research in biology, and is foundational in the field of evolutionary psychology.
Akçay doesn’t buy it. As a doctoral student at Stanford, he set out to review all the scientific literature on avian extra-pair parentage and found that fewer than half the published studies supported the “good genes” hypothesis. “But the theory was so dominant,” he recalls, “that even the papers that weren’t supporting it—that didn’t find evidence for anything like good genes—their discussions were full of excuses: Oh, this might be going on, or, That might be why we didn’t find it.”
Like a growing number of biologists, Akçay found the theory wanting on a number of levels. It provided little basis for deciding what counted as “good genes,” or outward signs of having them. Size? Plumage color? Song behavior? To some degree it was an arbitrary choice. And there was another problem. “If you do have good genes, there should be strong selection in favor of them,” he says. “So they should increase in frequency in the population very quickly [until] there aren’t any more ‘bad genes’ around.”
While it seemed that absentee male parents tended to be bigger and older than nest-bound males, the ultimate measure of fitness is survival, and Akçay’s 2007 meta-analysis found no difference in the survival rate of within-pair versus extra-pair offspring. The widely cited paper joined a growing literature questioning the “good gene” theory of sexual selection. These critiques—whose earliest versions are as old as Darwin’s theory, and are themselves hotly contested—have sparked attempts to refine or replace the theory of sexual selection. A great deal of Akçay’s subsequent research has been aimed at advancing an alternative explanation: that cooperation is at least as important as competition in the evolution of species.
In another paper, he proposed that extra-pair parentage in birds could arise from an “agreement” between territorial males. Perhaps males who were less successful in competition for territory were effectively striking a bargain with stronger ones. “We hypothesized that these weak males—they might be smaller or younger—were making arrangements with strong, more competitive males, that if they let them breed with a productive female, then the stronger males could have some of the offspring, some of the production from their nest. Sort of a side payment.” After all, birds were already accustomed to negotiating truces; they relied on something resembling an agreement about territorial boundaries to limit how much time and energy they spent policing borders, rather than, say, foraging for food.
Akçay, who came to Penn via the Evolution Cluster initiative in 2014, calls himself a “biological game theorist.” He’s interested in how complexity arises through evolution, and thinks cooperative game theory—a mathematical framework for analyzing interactions in which the behavior of multiple individuals affect each of their outcomes—is a uniquely useful tool.
“A lot of biological organization is predicated on having some sort of cooperation in place—between different organisms, between different cells, and so on,” he says. “A lot of the things that we think about when we think about evolution—competitive dynamics, predation, exploitation, and so on—without having some sort of cooperative interaction in place, they don’t even come up. Because a lot of exploitation, for example—a lot of cheating—is about trying to get the fruits of cooperation without paying the cost. So then, the question becomes, how does that cooperation evolve in the first place?—while not ignoring the fact that there are a lot of factors that can sort of unravel cooperation.”
As a theorist who typically collaborates with others rather than conducting experiments of his own, Akçay has tackled a wide range of subjects. He has proposed a model that explains the symbiotic relationship between legumes and rhizobia (the soil bacteria that help the plants fix nitrogen) through the lens of “biochemical negotiations” and “plant sanctions” at the level of the root nodules in which the bacteria establish themselves. With Taiwan-based biologist Sheng-Feng Shen, he has investigated the relationship between group size and social conflict in social animals and insects. He and his doctoral advisor, Stanford’s Joan Roughgarten, co-authored a somewhat controversial paper in Science arguing that Darwin’s theory of sexual selection should be replaced with a branch of game theory that focuses on cooperative dynamics, bargaining, and side payments. (Akçay’s theoretical work was featured in Roughgarten’s 2009 book The Genial Gene: Deconstructing Darwinian Selfishness.)
He teamed up with evolutionary biologist Elizabeth Pringle (currently a fellow at Germany’s Max Planck Institute for Chemical Ecology) to examine a three-party relationship between ants, scale insects, and Spanish elms, a tropical hardwood tree whose range extends from Mexico to Argentina. The trees host ant colonies that tend flocks of scale insects, which parasitically feed on the phloem running through the tree’s branches. These insects excrete sugar-rich honeydew that provides food for the ants, which in turn defend the tree’s leaves from herbivores. Pringle found that trees in drier, more stressful climates effectively invested more of their carbon resources into supporting their ant defenders than did trees buffered by wet climates—despite how costly the ants were to the trees during the prolonged dry season. Akçay developed a mathematical model that explained the phenomenon as a sort of “insurance” against the risk of extreme herbivory events; a vigorous defense army was especially crucial for trees that had a shorter wet season in which to grow energy-producing leaves, because they had less time in which to grow replacements for damaged ones. The mechanisms governing this investment strategy remain unclear, but the authors hypothesized that “trees can indirectly alter their investment by changing phloem chemistry, which affects scale-insect success and attractiveness to ants,” which could in turn lead the ants to expand their colonies.
Since landing at Penn two years ago, Akçay has spent a lot of time investigating population genetics and sociodemographic dynamics. He has joined Penn’s Social and Cultural Evolution Working Group, a departmentally diverse outgrowth of the Evolution Cluster led by linguistics assistant professor Gareth Roberts. With one of his post-doctoral students, he’s tackling the emergence of cultural traits that appear to be genetically maladaptive, such as the purposeful reduction of human fertility. His next paper presents a framework for understanding animal social networks primarily as a function of maternal bonding. He hopes that it will facilitate comparative studies of primate species, helping to uncover environmental or other variables that might influence social organization in predictable ways.
The common thread through it all, the biology of cooperation, would seem to run the length of the living world itself. And as the next section shows, understanding it better could lead to big rewards in applied science as well.
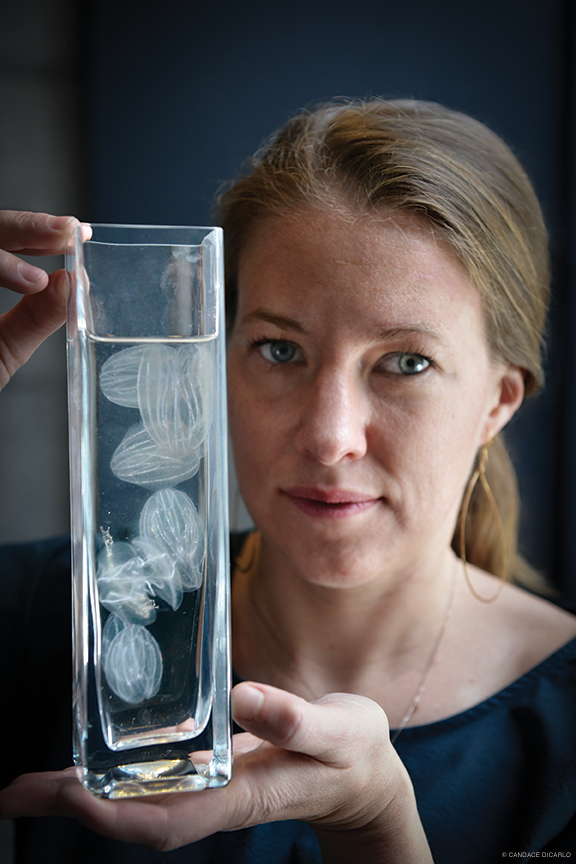
From Squid Camouflage to Alternative Energy in Three (Not So Easy) Steps
When Alison Sweeney started studying the biophysics of squid camouflage, it’s fair to say that she was interested in the same question that motivated her funder, the US Office of Naval Research. Namely, how did a certain family of squid manage, at a moment’s notice, to manipulate light waves to hide from predators in open water?
Sweeney, who is now an assistant professor of physics and astronomy—albeit one with a decidedly biological orientation—knew a thing or two about the underlying phenomenon, which is known as structural coloration. Structural color arises not from pigment but from microscopic structural patterns that reflect particular wavelengths of light while absorbing or scattering others. It pops up here and there in the animal kingdom. As a doctoral student at Duke, Sweeney had shown how heliconian butterflies use their iridescent wings, which polarize light, to recognize mates. Squid tissue is capable of similar light-bending tricks—but with one very important difference: it’s alive.
“When you see iridescence or structural color in other places,” Sweeney says, “it comes from something that’s dead. So, butterfly wings have hair-like scales on them—those are dead. Beetle carapaces are essentially a dead part of beetle skin.” The same goes for iridescent bird feathers, which, like human hair, are primarily composed of dead cells.
Sweeney’s squid owed their iridescence to specialized living cells known as iridocytes, which essentially churn out dense bricks of protein and orient them in light-scattering patterns. As she investigated the cellular mechanisms governing this finely tuned process, she couldn’t shake a more basic question: “Where the heck did these things come from in the first place?” And exactly what sort of cell was an iridocyte? “Is it like a mucus cell, or a skin cell, or what?”
Her search for an answer steered her toward a phenomenon considerably more mysterious than squid camouflage.
Like a latter-day Carl Linnaeus, she made a list of every organism she could find that exhibited living-cell iridescence. It turned out that there were a fair number—but they had one thing in common: they were all mollusks.
Yet pinning an evolutionary history on the trait hardly demystified it. In some ways, it made it even stranger. Mollusks comprise a huge phylum. On one end are mobile swimmers like squid and octopuses, which actively hunt prey, flee from predators, and mate with one another. On another end are bivalves, like oysters and clams, that spend their entire lives rooted in a single spot, and reproduce more like pine trees do—by means of drifting clouds of sperm and eggs that unite (or don’t) at random.
It was easy to see how free-swimming mollusks were using iridocytes: to hide, or send signals. But one of the most conspicuous examples of molluscan iridescence was baffling: giant clams, whose lips produced gaudy, psychedelic displays of color that defied explanation.
“Typically when evolution invents iridescence, it’s to make a signal,” Sweeney explains. “Butterflies and birds signal to each other. There are also examples of iridescent camouflage. So you can signal to predators, or to mates, or you can be camouflaged—and I had a hard time coming up with any other example of a use for iridescence. And here we had this clam that was brightly colored, doesn’t actively mate with anything, and is just open for the taking by any predator that wants to come grab it.”
She continued working on the squid, but the clams kept nagging her. When an opportunity came up to assist a coral-spawning expedition in the South Pacific, she signed on, hoping to get up close and personal with a genus of football-sized clams known as tridacnids.
What she discovered was a system that had harnessed optical physics to solve a problem that has bedeviled alternative-energy scientists for decades. The clam’s iridocytes weren’t for signaling or hiding. They acted “like an electrical transformer,” Sweeney realized, “but instead of a transformer for electricity, a transformer for light.”
Electrical transformers—those gray cylindrical boxes on power poles—convert high-voltage electricity running through a wire (which is efficient but dangerous) into safer low-voltage current that’s better-suited for household use. The iridocytes did something analogous. The clue to their function lay in the vertical pillars of algae living beneath them, symbiotically embedded in clam tissue. Like most algae, these made their living off sunlight. But the intensity of tropical sunlight posed a danger to them: too much direct exposure would cause harm.
That’s where the clam’s iridocytes came in. They admitted the wavelengths of light best suited for photosynthesis, reflecting them down into the tissue at a particular angle, while dispersing the rest—which is what gave the clams their wildly colorful appearance.
“So, taking energy at a rate or intensity that is dangerous, and basically spreading it out into an intensity that the algae can use—while not losing any of that energy—is essentially what the clam tissue does,” Sweeney explains. “And it just so happens that the geometry of the algae pillars matches the rate of spread coming out of the iridocyte. And then everything works out so that the new light level that the algae see in the pillars is the correct dose for the algae to use efficiently without being damaged.” By keeping the algae happy, the clams got something, too: a portion of the energy-rich nutrients the algae produced.
For years, scientists have recognized the potential of algae to produce biofuels. On the level of a single organism, it makes a lot of sense. But scaling up has been a challenge.
“The easiest way to grow algae is essentially in a bucket,” as Sweeney puts it. “And it doesn’t really matter how fancy you make your bucket, it’s going to suffer from the same problem that the clam actually solved, which is that there’s going to be very bright sunlight at the top of your bucket, and very little sunlight very quickly underneath that. So you can’t efficiently fill your bucket with algae, because you have this problem of light penetration. Everybody at the surface is super-stressed, and everybody beneath is in the dark.”
You can try to even things out by stirring the bucket, but that siphons off too much of the energy you’re trying to produce.
“The clam has evolved a completely surface-area-efficient unstirred photo-bioreactor—a mechanism to grow as many algae as possible in a given surface area without having to stir the system,” Sweeney says. “So it’s neat to think that you could build a device like this.”
Since coming to Penn in 2012, as one of the first faculty members recruited through the interdisciplinary Evolution Cluster initiative, Sweeney has been trying to do just that with Shu Yang, a professor of materials science and engineering who has a secondary appointment in chemical and biomolecular engineering.
“She’s the master of biomimicry in nanofabrication,” Sweeney says. “We have a vision of something that would look sort of like a solar panel, but instead of making electricity directly from sunlight, they’d be tiled with clam-like material that had about four millimeters of algae growing on top of it.” They are trying to make synthetic iridocytes, and investigating strategies to create “some sort of gel-like material you could stretch over a panel that would keep the algae in place to culture them.”
Meanwhile, she’s collaborating with Seth Hurzon, a synthetic organic chemist at Yale, to mimic another tantalizing aspect of the clam system.
“Bioengineers have worked really hard to figure out how to short-circuit plant metabolism to kick out molecules that might be useful as fuels,” she says. The tridacnid clams have evolved their own solution. “There’s a chemical cue coming from the clam, so that literally when the algae smell clam, they short-circuit their metabolic pathways and put 40 percent of their photosynthesized carbon into glycerol, which is a small three-carbon molecule that readily oozes out of the algae and into the clam.” She and Hurzon are trying to identify the chemical scent by which the clam accomplishes this feat. If they’re lucky, it could end up being “some small organic molecule that you could imagine synthesizing and doping your algae with.”
If the projects bear fruit, the cargo ships and passenger planes of the future might just be fueled by fuel-secreting algal solar panels instead of crude oil. Meanwhile, Sweeney is still working on squid camouflage, trying to figure out how the iridocytes actually assemble themselves within the animal’s tissue. It’s a challenge that particularly appeals to her graduate physics students.
“Clam optics is passé if you’re a physics grad student,” she laughs. “It turns out that self-assembly is the sexy topic.”
The Office of Naval Research, on the other hand, is now interested in both.